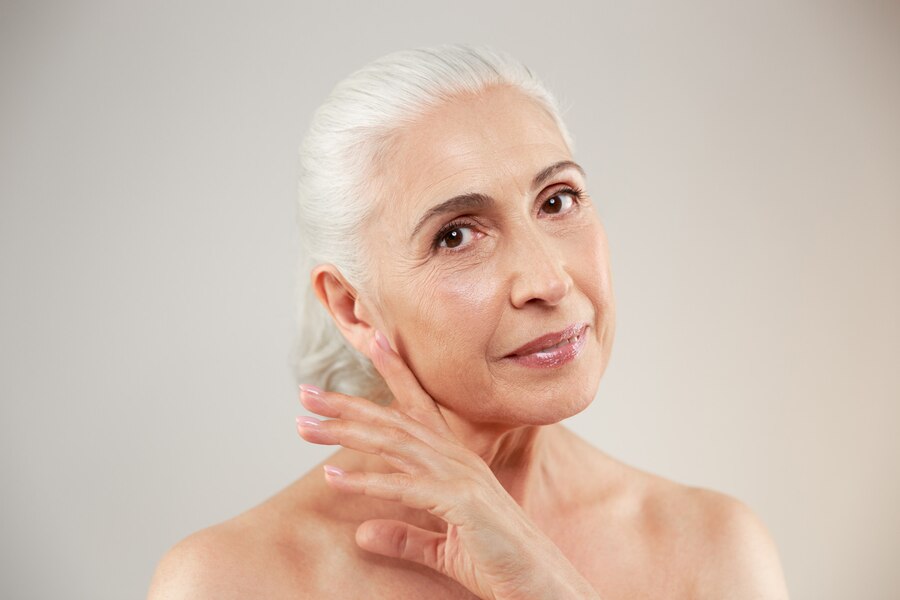
Autophagy is a process of cellular self-degradation in which portions of the cytoplasm are sequestered within cytosolic double-membrane vesicles and delivered to the lysosome/vacuole. This process occurs in all eukaryotic cells and is partly a stress response; autophagy is induced during starvation and hypoxia. However, autophagy also plays a role during development and is associated with a range of diseases. Accumulating data also suggest the involvement of autophagy in aging. For example, the role of various hormones and nutrient sensing pathways in life span extension may involve autophagy. Similarly, autophagy is the primary mechanism for removing damaged organelles, such as mitochondria, which may have a direct impact on aging. Here, we review the role of autophagy, with an emphasis on the signaling pathways that are involved in regulation, and the consequences of autophagy induction with regard to aging.
Autophagy, an evolutionarily conserved catabolic pathway in eukaryotic cells, plays an important role in stress response and the degradation of damaged cytosolic components (94). There are three general types of autophagy that can deliver intracellular materials into lysosomes for degradation: macroautophagy, microautophagy, and chaperone-mediated autophagy (CMA) (209). CMA is restricted to a subset of proteins bearing a particular pentapeptide motif (39). Furthermore, CMA involves the translocation of unfolded substrates across the lysosome membrane and, therefore, is unable to accommodate large protein aggregates or organelles. Unlike CMA, both micro- and macroautophagy involve dynamic membrane rearrangements resulting in the ability to enwrap large structures, such as organelles. Microautophagy refers to the sequestration of cytosolic materials through direct invagination of the lysosomal membrane. In contrast, during macroautophagy, double-membrane cytosolic vesicles, termed autophagosomes, sequester the cytoplasm and deliver this cargo to the lysosome/vacuole for degradation (209).
Although macroautophagy is mainly considered a bulk sequestration process, selective autophagy to degrade damaged or excess organelles, such as mitochondria (mitophagy) (110), peroxisomes (micropexophagy and macropexophagy) (50), endoplasmic reticulum (ER; reticulophagy) (210), parts of the nucleus (piecemeal microautophagy of the nucleus) (158), and even ribosomes (ribophagy) (97) also have been reported. Most of the autophagy-related pathways mentioned above share similar molecular components and morphological features. In addition to catabolic degradation, in Saccharomyces cerevisiae, Pichia pastoris, and Candida albicans, a biosynthetic pathway, the cytoplasm to vacuole targeting (Cvt) pathway, also uses the autophagy machinery to deliver the vacuolar hydrolase aminopeptidase I (209). A schematic diagram of autophagy-related pathways is shown in FIGURE 1.

Download figureDownload PowerPoint
The autophagy process can be dissected into several steps: induction, vesicle nucleation, cargo sequestration, autophagosome expansion and completion, vesicle fusion with the lysosome/vacuole, degradation, and nutrient recycling (70, 113). The identification of many autophagy-related (ATG) genes provides insight into the molecular machinery of the autophagy process. Among 31 ATG genes that have been identified so far, the majority of them function at the step of autophagosome membrane formation. Depending on their function, the Atg proteins can be classified into different groups: The Atg1 protein kinase complex is involved in autophagy induction and also acts along with Atg2 and Atg18 to regulate the cycling of the integral membrane protein Atg9; Atg14 is part of a phosphatidylinositol 3-kinase complex that is required for vesicle nucleation; the Atg8 and Atg12 ubiquitin-like conjugation systems are involved in autophagosome formation, and Atg8 controls the size of the autophagosome; and Atg9, Atg23, and Atg27 are thought to be part of the complex that delivers membrane needed for autophagosome production (70, 108, 203, 204).
Owing to the increasing understanding in the biological functions of autophagy, the significance of this process in diseases, aging, and immunity has been recognized (70). Among the different functions of autophagy that have been studied, growing evidence supports a role of autophagy in life span extension. For example, mutations that decrease mitogen-responsive pathways, such as in the insulin/insulin-like growth factor 1 (IGF-1) receptor and target of rapamycin (TOR), as well as a reduction in food intake, are known to increase life span from yeast to mammals, although the mechanisms of these pathways in aging regulation is not clear (9). Results from various studies indicate that autophagy may play an important role as a downstream effector of these life span regulatory pathways. One focus of this review is, therefore, the interaction between macroautophagy (referred to as autophagy hereafter) and several distinct longevity regulatory pathways. In addition, mitochondrial respiration and alteration have been linked to aging (170). Mitochondria can regulate autophagy through the generation of ROS. The role of this autophagy induction, however, can serve as an important intervention for either mitochondrial turnover to maintain homeostasis or as a signal for autophagy-associated cell death.
Autophagy and Aging
Aging cells gradually accumulate various deleterious changes, resulting in a decline in cellular function and eventually leading to cell death and disease. The accumulation of damaged macromolecules and organelles is one of the most persistent changes in aging cells (185) and is associated with the decline of different catabolic pathways (36). Autophagy deficiency during aging has been proposed to be the main cause of this biological “waste” accumulation (35). In support of this theory, it has been found that loss-of-function in autophagy genes resulting in intracellular accumulation of damaged proteins and organelles in mice, accelerates aging and life span shortening in Caenorhabditis elegans and Drosophila melanogaster (77, 98, 128). In contrast, elevating autophagy activity increases life span in Drosophila and rescues aged cells from accumulating dysfunctional mitochondria (175). Moreover, the failure in the clearance of proteins due to the decline of autophagy is associated with age-related pathogenesis in neurodegenerative diseases. For example, the conditional knockout of Atg5 or Atg7 in the nervous system causes neurodegeneration in mice (61, 95). Similarly, the Drosophila p62 homolog Ref(2)P, which is associated with neural aggregates and inclusion bodies found in various neural degenerative disorders (214), accumulates in the brain of autophagy-defective young flies (141). Thus autophagy plays a cytoprotective role in preventing the accumulation of disease-causing proteins. However, the fact that autophagy is associated with cell death under certain settings might suggest its involvement in different aspects of aging. For instance, overexpression of autophagy genes or inducing autophagy in apoptosis-deficient cells can lead to cell death (114). It is important to point out, however, that the latter situations may be nonphysiological. There is at present little, if any, direct evidence that autophagy normally causes cell death. Instead, it is likely to act primarily as a cytoprotective response.
Signaling, Aging, and Autophagy
Results from different organisms indicate that decreasing the activity of conserved nutrient-sensing signaling pathways, including insulin/IGF-1, TOR, protein kinase A (PKA), and protein kinase B (PKB), can extend life span. This phenomenon suggests that the conserved nutrient responsive pathways can affect life expectancy in evolutionarily diverse species. Interestingly, autophagy is sensitive to nutrient availability and is negatively regulated by these signaling pathways. The link between autophagy and nutrient-sensing pathways that regulate life span has been demonstrated in many studies.
The insulin/IGF-1 pathway
In C. elegans, several long-lived mutants carry gene mutations that correspond to the components of the insulin/IGF-1 signaling pathway. For instance, mutations in daf-2, encoding the insulin/IGF-1 receptor, age-1, which encodes the catalytic subunit of the phosphatidylinositol 3-kinase (PtdIns3K), and pdk-1, which encodes phosphoinositide-dependent protein kinase 1 (PDK1), extend life span (90, 130, 147). The longevity regulated by this pathway is through attenuation of insulin/IGF-1, the downstream PtdIns3K signaling, and in part by the upregulation of downstream stress response genes through the forkhead FoxO-related transcription factor (DAF-16) (105). Loss-of-function in DAF-18, a homolog of the phosphatase and tensin homolog on chromosome 10 (PTEN), de-suppresses the activity of PtdIns3K, thus inhibiting the longevity phenotype of daf-2 and age-1 mutants (101) (FIGURE 2).

Download figureDownload PowerPoint
Similar life span extension effects also appear in Drosophila. Flies with loss-of-function mutations in the insulin-like receptor (183), or its receptor substrate (chico) (31), or gain-of-function in a FoxO-like transcription factor (72) result in significantly increased life span. However, the longevity effect in mammalian systems is rather ambiguous, probably due to the complexity of the metabolic pathways. A variety of mutations downregulating insulin-like signaling activity can extend mammalian life expectancy. Mice heterozygous for the IGF-1 receptor, as well as dwarf mice with mutations in Pit1dw, Prop1df, and Ghr genes, are long-lived and have reduced plasma insulin and/or IGF-1 (13, 16, 33). In contrast, the variable effects on life span in mice with tissue-specific loss-of-function mutations in the insulin receptor and the developmental and multiple endocrine defects in IGF-1-deficient animals complicate the interpretation of the data on the role of IGF-1 in aging (13). It is interesting to note that a recently identified aging suppressor gene, Klotho, in mice can block insulin-mediated glucose uptake, suppress the phosphorylation of insulin and IGF-1 receptors, as well as repress already activated insulin receptors, thus resulting in increased longevity (99). Klotho mice show better oxidative stress resistance at the cellular and organismal level by inducing manganese superoxide dismutase (MnSOD) expression through activation of stress response FoxO forkhead transcription factors (206). The increasing resistance to oxidative and metabolic stress by upregulating FoxO transcription factors in long-lived mutant animals is thought to contribute, at least in part, to the long life span. Growing evidence shows that mammalian forkhead family transcription factors influence the life span of stem cells, control stress response gene expression, and have tumor suppressor functions (6).
It has been known for years that autophagy can be induced by low insulin levels during fasting, whereas high insulin levels are suppressive (137). However, the link between insulin/IGF-1 in longevity and autophagy is not clear. Several lines of evidence suggest that autophagy is involved in aging regulation through the insulin/IGF-1 signaling pathway. Depletion of C. elegans BEC-1, whose counterpart is yeast Atg6 and the human tumor suppressor Beclin 1, inhibits the increased longevity phenotype in the daf-2 mutant, and slightly shortens the life span of wild-type animals (128). Similarly, knocking down ATG7 and ATG12 through double-stranded RNA (dsRNA) partially suppresses the life span extension in daf-2 mutants and causes significant shortening of the mean but not the maximum life span of wild-type worms (64). Furthermore, bec-1, atg-18, and lgg-1(LC3/Atg8) mutants partially inhibit the increased longevity of daf-2 mutants (188). Theses results suggest that autophagy and other parallel pathways may regulate life span through insulin/IGF-1 signaling. For example, the ubiquitin-proteasome system and the heat shock factor HSF-1, which controls stress-inducible gene expression and protein folding homeostasis, is also required for the life span extension of daf-2 mutations (136).
The downstream insulin/IGF-1 components, fork-head transcription factors, also regulate autophagy activities. Accordingly, activation of FoxO in the Drosophila fat body induces autophagy in feeding larvae, whereas a null mutation of FoxO decreases starvation-induced autophagy (78). Similarly, FoxO3, the closest mammalian ortholgue of daf-16, which is associated with the long-lived phenotype of daf-2 mutants in C. elegans as discussed previously, is necessary and sufficient to induce autophagy in skeletal muscles (122, 123). Thus autophagy stimulation following a decrease in IGF-1-PtdIns3K-Akt signaling can occur in a FoxO3-dependent manner. All together, these findings imply that autophagy can function downstream of insulin/IGF-1 to regulate life span (FIGURE 2).
TOR
The insulin/IGF-1 pathway negatively regulates autophagy through the target of rapamycin (TOR), which also plays an important role in aging (75). TOR is a highly conserved serine/threonine protein kinase that regulates cell growth and proliferation in response to growth factors, nutrient signals, and energy status (143). There are two functionally distinct TOR complexes in yeast, TORC1, which is rapamycin sensitive and regulates cell growth, translation initiation, and responses to nutrients, and TORC2, which is rapamycin insensitive and regulates cytoskeletal remodeling (118). Inactivation of TOR signaling results in several physiological characteristics of starvation, including activation of autophagy (161). In yeast, TORC1 controls autophagy though Atg1, Atg13, and Atg17 (29, 82). Inactivation of TORC1 by rapamycin treatment or nitrogen starvation alters the phosphorylation state of Atg13, possibly facilitating an interaction with Atg1 kinase, which may induce autophagy (82).
There are two types of aging in yeast that can be used as models for higher eukaryotes: the replicative life span (RLS), which is measured by the number of daughter cells that a mother cell can produce before senescence (81), and chronological life span (CLS), which is determined by the length of time a non-dividing cell can survive (156). The yeast RLS has been suggested to be a model of aging for mitotically active cells, whereas the CLS has been modeled as postmitotic cell aging (120). Defects in TORC1 signaling have been shown to increase yeast life span both replicatively and chronologically (14, 81). Long-lived animals with mutations resulting in decreased TOR signaling have also been reported in C. elegans (193). Decreasing TOR signaling by RNAi knockdown of TOR, let-363/CeTor, or the TOR accessory protein raptor, daf-15, extend adult life span (193). In C. elegans, the longevity effect of TOR deficiency is mediated by autophagy based on the finding that mutations in bec-1, unc-51 (yeast Atg1 ortholog), and atg-18 completely abolish the long-lived phenotype seen in let-363/CeTor RNAi-treated animals (188). In Drosophila, overexpression of an upstream TOR inhibitor (Tsc1-Tsc2), a downstream effector, dS6K (which may act through a feedback loop to suppress TOR), or dominant negative forms of dTOR extend life span (84). A recent study in yeast further suggests that autophagy is required for the life span extension in TOR signaling-deficient mutants (81) (FIGURE 2).
Amino acid starvation increases CLS in yeast through reduced TOR signaling (156). For instance, Gln3, a GATA transcription factor controlled by TOR, upregulates many amino acid permeases, integral membrane proteins that transport specific amino acids across the membrane, and amino acid biosynthetic enzymes. Deletion of several Gln3-regulated permease genes, such as the general amino acid permease Agp1, increase yeast CLS. Deletion of amino acid- and nitrogen-acquisition proteins may limit the concentration of cellular amino acids and increase life span by downregulating TOR signaling (156). In higher eukaryotes, decreasing cellular amino acid levels by either reducing the dietary source or reducing uptake in permease or transporter mutants also promotes longevity though reducing TOR signaling (132, 154).
Evidence supporting the interaction between TOR and insulin/IGF-1 pathways to control life span comes from genetic studies of C. elegans and mammalian systems (75, 193). The life span of daf-2 nematodes is not further extended with RNAi knockdown of let-363/CeTor. These two mutants share similar phenotypes other than life span extension. However, unlike daf-2 animals, the life span extension of let-363/CeTor does not require the activity of the forkhead transcription factor DAF-16, indicating that TOR may act downstream of, or in parallel with, DAF-16 (41). In worms, the transcription of DAF-15, the Raptor-like regulatory TOR interacting partner, is inhibited by DAF-16, indicating that TOR may act downstream of DAF-16 (41, 75).
RAS/PKA
In addition to TOR, Ras/cAMP-dependent protein kinase A (PKA) also regulates autophagy in various organisms (19). The RAS/PKA pathway plays an important role in cell proliferation, stress response, and longevity (186). In yeast, in response to nutrient-rich conditions, two redundant Ras GTPases, Ras1 and Ras2 (RAS), activate adenylate cyclase (encoded by the CYR1 gene) to produce cAMP. Binding to cAMP allows the dissociation of the PKA regulatory subunit, Bcy1, activating the PKA catalytic subunits, Tpk1, Tpk2, and Tpk3 (155). In a study using a sequence-based proteomic approach to identify PKA substrates in S. cerevisiae, three autophagy-related proteins were identified: Atg1, Atg13, and Atg18. These proteins act at an early step of autophagy, which agrees with the observation that activation of Ras/PKA inhibits early in the process (18). Constitutive activation of PKA by depleting its regulatory subunit Bcy1 suppresses autophagy induction during nutrient-depletion and can override the inactivation of TORC1, indicating that PKA acts as a negative regulator for autophagy (18, 211) (FIGURE 2).
Mutations that result in attenuation of Ras/PKA signaling, such as in ras2Δ, increase both the RLS and CLS in yeast strains (48, 119). This CLS increase requires the downstream effectors Msn2 and Msn4 (49, 197). Nutrient depletion would first activate Rim15, a glucose-responsive kinase that integrates and transduces TOR, PKA, and Sch9 signals in response to nutrients. Active Rim15 upregulates the expression of the stress-response transcription factors Msn2 and Msn4, which subsequently results in the transcription of genes involved in stress response, such as superoxide dismutase (SOD) (21). However, many studies suggest that MSN2, MSN4, and RIM15 have opposite effects on RLS. Similarly, in SOD mutants, the RLS is shortened. One possible explanation is that the stress-resistance genes that contribute to the CLS may affect the budding process, thus reducing the RLS (178). Ras/PKA also regulates the life span in mammals based on the observation that type 5 adenylyl cyclase knockout (CA5-KO) mice live 30% longer than their wild-type littermates (208). In the CA5-KO mice, the growth hormone level is decreased, suggesting that the insulin signaling in these mice is also decreased. Given the fact that attenuation of Ras/PKA signaling is associated with longevity and that Ras/PKA negatively regulates autophagy, it can be speculated that the longevity effect of Ras/PKA may be mediated through autophagy. However, the link between autophagy and Ras/PKA in life span regulation still needs to be tested.
PKB/Sch9
Sch9 is a serine/theronine protein kinase, and its kinase domain is homologous to yeast PKB, C. elegans AKT-1, AKT-2, and mammalian protein kinase B (PKB/Akt) (34, 49). Sch9 is also involved in nutrient sensing, oxidative stress resistance, and cell size control (76). PKA and Sch9 may function in parallel, and they share many targets to control several physiological events, such as growth, cell cycle progression, and glycolysis (119, 186). In yeast, similar to Ras/PKA signaling mutants, transposon insertional sch9 mutants show CLS that is three times longer than the wild type. Life span extension in sch9 mutants as well as mutants defective in PKA is mediated by the serine/threonine kinase Rim15 and the downstream stress resistance transcription factors Msn2/4 (197).
Sch9 is a negative regulator for autophagy. Hyperactivation of Sch9 partially suppresses autophagy when TOR is inactivated by rapamycin. Inactivation of both PKA and Sch9 without inactivating TORC1 can induce autophagy, and inactivation of all three has an additive effect, indicating that these factors may, at least in part, regulate autophagy in parallel (211). Msn2/4 and Rim15 are involved in PKA- and Sch9-mediated autophagy regulation (211). Although there is no direct evidence, it is reasonable to link the longevity phenotype of PKA and Sch9 signaling-attenuated mutants with autophagy activation. Future experiments are needed to elucidate the interaction between autophagy and PKB-regulated life span extension.
PKA, Sch9, and TOR may control some cellular processes in concert. Indeed, these kinases integrate nutrient signaling and regulate cell growth (152, 162, 166). A functional interaction between the corresponding pathways has been suggested because of the overlapping downstream targets and the similar phenotypes in cells deficient in either pathway (177, 200). Additionally, these pathways, along with insulin/IGF-1 signaling, have been linked to life span regulation in diverse species as discussed above. This supports the idea that decreased activity in conserved nutrient sensing pathways extends life span, that this is a common phenomenon in diverse species, and that autophagy may act downstream of these pathways to accomplish this beneficial effect (156).
LKB1-AMPK
AMPK functions as an energy sensor. In response to metabolic stress conditions, AMPK activates several cellular processes to restore normal energy levels, such as glucose uptake and glycolysis (62). AMPK functions to extend life span in C. elegans (4) and to promote autophagy in human cell lines (115). Paradoxically, in Zmpste24-null mice, a model of human Hutchison-Gilford progeria characterized by premature aging, there is an extensive basal activation of autophagy due to the activation of LKB1-AMPK and inhibition of TOR (124). It is possible that the nuclear structure abnormalities in these progeroid mice are due to a defect in lamin A maturation, which may through an unknown mechanism trigger a series of anti-aging responses, such as the use of alternative metabolic pathways and the induction of autophagy activity. However, this adaptive response is unable to compensate for the cellular defects, which then lead to the chronic activation of autophagy. This chronic autophagic response may overcome its beneficial anti-aging effects, resulting in premature death of the mutant mice (124).
Calorie Restriction
Calorie restriction (CR) refers to the limiting of dietary energy intake while maintaining essential nutrient levels. In various species, CR has been shown to extend life span, slow aging, and delay the onset of age-associated diseases, such as cancer, diabetes, neurodegeneration, and cardiovascular diseases (125). For instance, CR suppresses several forms of radiation- or carcinogen-induced cancers in rodents (55). In humans, CR lowers cholesterol, blood pressure, blood glucose, and plasma insulin (66). In mice, a 30–50% reduction of caloric intake increases the average and maximum life span 20–50% compared with ad libitum fed control mice (198). The beneficial effects of CR in primates are the improvement of aging biomarkers, such as blood pressure (163, 164). A study of an Okinawan population shows that CR may contribute to average and maximum life span extension and lower the risk of age-related chronic diseases (199).
How does CR work? CR causes the gene-expression profile to shift toward increasing protein turnover, damage repair, and stress response while attenuating age-associated gene induction (102, 103). Insight may come from long-lived mutants carrying mutations in conserved nutrient-responsive pathways: insulin/IGF-1, TOR, PKA, PKB/Sch9. As discussed previously, mutations that cause the attenuation of these pathways have similar effects on life span increase as that of CR. A recent study indicates that the TOR and PKB/Sch9 signaling pathways may mediate the life span regulation of CR (81, 116, 197). Removal of either asparagine or glutamate from the media induces a starvation response and increases survival through inactivation of TOR (156). Moreover, CR fails to further increase yeast RLS seen in tor and sch9 deletion mutants, indicating the involvement of these two pathways in CR-mediated RLS extension (79, 81).
CR reduces plasma insulin levels; however, the involvement of the insulin/IGF-1 pathway in CR-mediated longevity remains in debate. Exposure of daf-2 mutant worms to CR conditions further extends life span, suggesting that CR and the insulin/IGF-1 signaling pathway may function in parallel to regulate aging (69). Life span extension by reducing glycolysis requires DAF-16 (60, 106), and decreased glucose metabolism is a common feature in CR and mutants attenuated in TOR, PKA, PKB/Sch9, and insulin/IGF-1 signaling activities (41). The above result links the insulin/IGF-1 pathway to the metabolic changes associated with CR, but the connection between CR and the insulin/IGF-1 signaling pathway is more apparent in flies and mice. CR does not further extend life span in chico mutant flies (carrying a mutation in the insulin receptor substrate) and long-lived growth hormone receptor/GH-binding protein knockout (GHRKO) mice, indicating that the insulin/IGF-1 pathway may mediate the CR effect (15, 33, 149).
CR and autophagy
CR may reduce the activity of TOR, PKA, and PKB/Sch9 signaling and as a result decrease protein synthesis and metabolic changes (87). Decreasing these nutrient responsive pathways may induce autophagy. Since the degree of autophagy activity is responsive to the nutrient levels, it is logical to speculate that autophagy might be induced under CR conditions (98, 135). Indeed, CR maintains autophagy activity at juvenile levels and reverses the age-related decline of autophagy activity in rat hepatocytes and cardiomyocytes (201). Some data suggest that the effects of CR in longevity are mediated through reducing TOR activity and activation of autophagy in organisms from yeast to mammals (59, 96, 188). For example, autophagy is induced in eat-2 (eating-defective-2) worms, where the function of the feeding organ has been disrupted, which show increased life span, supporting the idea that autophagy induction is coupled with CR-mediated longevity (188). The knockdown of bec-1 and atg7 by RNAi treatment suppresses the long-lived phenotype associated with CR. Moreover, loss of function in bec-1 and unc-51 (yeast ATG1) completely inhibits the increased longevity of the eat-2 mutant, indicating that autophagy is required for the CR-mediated longevity (188).
Sir2
In addition to the conserved nutrient sensing signaling pathways, the sirtuin gene family that functions in maintaining genome stability is also found to regulate aging, although the relationship between CR and sirtuin genes as well as their effect on aging remain controversial. The name sirtuin comes from the yeast protein silent information regulator 2 (Sir2), the first member identified in this family. Through a screen, the SIR complex was identified as a regulator for RLS (86). In yeast, Sir2 activity is required for the longevity effect of CR (57). SIR2 encodes a histone deacetylase that was identified to be involved in silencing the mating type loci (HML and HMR), telomeres, and ribosomal DNA (rDNA) locus RDN1 (54). The Sir2-associated RLS extension mainly occurs through suppressing the recombination of repetitive rDNA and the formation of extra-chromosomal circular forms of rDNA (54, 176). The histone deacetylase activity of Sir2 is nicotinamide adenine dinucleotide (NAD+)-dependent, which allows the regulation of its activity depending on metabolic status and changes in the NAD+/NADH ratio. CR may increase the NAD+ ratio, thus increasing Sir2 activity to promote longevity (187). However, beneficial effects of CR still can occur in yeast defective in respiration (79).
The link between Sir2 and CR has been reported, although some studies are in conflict regarding the role of Sir2 in life span extension by CR (87). In the case of yeast, Sir2 activity correlates with yeast RLS under CR conditions. Deletion of SIR2 shortens the yeast RLS, whereas elevation of its activity leads to longevity (80, 116). However, SIR2 deletion does not shorten the CLS (47). Overexpression of Sir2 in worms (sir-2.1) extends life span by up to 50%. Sir-2.1 functions upstream of DAF-16 in the insulin/IGF-1 signaling pathway (187). In adult worms, complete starvation increases life span to a greater extent than CR. This type of life span extension is independent of insulin/IGF-1 signaling and Sir-2.1 (59). Drosophila Sir2 is required for the life span-extension effect of CR (160). In Sirt1 (mammalian Sir2 ortholog) knockout mice, the physiological and behavioral pattern associated with CR does not occur, suggesting that Sirt1 mediates the effects of CR (24). The Sirt1 activator resveratrol activates Sirt1 and extends life span in mice fed with a high-calorie diet and in fish (7, 190). Furthermore, Sirt1 activates fatty acid mobilization from white adipocytes under CR in 3T3-L1 adipocytes. Upregulation of Sirt1 stimulates lipolysis and reduction in fat, which is sufficient to extend life span in mice (153). On the other hand, results in some types of mammalian cells and tissues suggest that Sirt1 might accelerate aging. Sirt1−/− mice embryonic fibroblasts (MEFs) extend replicative life span that is correlated with increasing proliferative capacity under oxidative stress (30). Evidence also suggests that Sir2/Sirt1 is part of the regulation loop in the insulin/IGF-1 pathway. Forkhead transcription factors are targets of Sir2, and in worms Sir2 modulates the activity of DAF-16 (187) (FIGURE 2). Similarly, mammalian Sirt1 deacetlyates FoxO transcription factors (17, 37). Moreover, Sirt1 represses IGF binding protein 1 expression. Sirt1-null mice have increased production of IGF binding protein 1 and show several phenotypes correlated with long life span (111). In addition to Sirt1, among seven mammalian Sir2 homologs, some may have similar functions to Sir2. For instance, Sirt6 binds to chromatin and suppresses genome instability by enhancing resistance to DNA damage, and Sirt6 knockout mice show accelerated aging phenotypes and age-associated pathology (138).
A recent study demonstrates the ability of Sirt1 to induce autophagy. Overexpression of Sirt1 induces autophagy in both normal growth and starvation conditions, but not in the dominant negative sirt1 mutant, suggesting that Sirt1 alone is sufficient to induce autophagy even in the presence of nutrients (104). Atg5, Atg7, and Atg8 are deacetylated by Sirt1 in an NAD+-dependent manner, and the acetylation levels are reduced under starvation conditions, suggesting that Sirt1 can adjust autophagy activity to match the metabolic status (104). In sirt1−/− mice, embryonic heart tissues and neonatal tissues within the first 3 h after birth show increased p62 levels, which serves as an in vivo marker for impaired autophagy (12, 104). Some phenotypes of sirt1−/− closely resemble atg5−/− mice, such as the accumulation of abnormally shaped mitochondria, early mortality, and abnormality of energy-responsive pathways (98, 104). Moreover, mammalian Sirt1 has a cellular protective role against certain forms of neurodegeneration (5). Enhanced Sirt1 might mimic CR conditions to induce autophagy, which then promotes damaged protein and organelle removal to protect against age-related diseases. Future studies are needed to elucidate the link between sirtuin-mediated life span extension and the regulation of autophagy.
ROS, Mitochondira, Aging, and Autophagy
Mitochondria and aging
Accumulation of mitochondrial DNA (mtDNA) mutations as well as a decline in mitochondrial function is a common feature in aging cells (196). Studies performed in humans reveal a decline of ATP production with age (174). Similarly, some age-related diseases are associated with mitochondrial alterations. For instance, hearing loss in aged animals is associated with mitochondrial dysfunction and mtDNA mutations (207). Mitochondria are the primary source, and a target, of reactive oxygen species (ROS), and the production of ROS is proposed to play an important role in aging (63). The mitochondria theory of aging, a variant of the free radical theory of aging, proposes that ROS production during respiration causes accumulation of oxidative damage in mitochondria and mtDNA, which would eventually lead to aging and death (63). Electron leakage at the electron transport chain (ETC) during respiration, through both detoxification enzymes and non-enzymatic reactions, can generate ROS. ROS cause oxidative damage to mtDNA and inhibition of the ETC, resulting in even higher levels of ROS production. This “vicious cycle” in the end leads to cellular function decline and aging (1).
According to the mitochondria theory of aging, one would assume that the rate of aging would correlate with the ROS level produced at mitochondria. Additionally, reducing ROS production or decreasing respiration might retard the aging process. Nevertheless, evidence both supports and contradicts the ROS theory of aging as shown in Table 1 and Table 2. Furthermore, respiration rate and ROS production are not proportional to each other (14). Instead, ROS production is determined by the state of the ETC (196). For example, in long-lived fly mutants expressing human uncoupling protein UCP2 in mitochondria of fly neurons, there is increased respiration, enhanced oxidative stress resistance, and decreased ROS production (53). Life span extension is observed in mutants displaying both increased and decreased respiration, and mild increases in ROS are seen in long-lived mutant flies and worms with partial disruption of the mitochondrial ETC (133, 157).
A class of long-lived mutants, Mit, in C. elegans paradoxically display mitochondrial dysfunction, and most of the affected genes encode components of the ETC (3, 107, 139). The mechanism attributed to the life span extension has been the subject of intense study. The life span of long-lived respiration-defective nematodes is further increased by a mutation attenuating the insulin/IGF-1 pathway (40). This additive effect on life span extension suggests that the insulin/IGF-1 signaling pathway and mitochondrial respiration machinery function in parallel in life span regulation. Several hypotheses have been proposed to explain the possible metabolic strategies that could account for the long life span. One of the intriguing hypotheses is that autophagy is required for the longevity of Mit mutants (194). In long-lived mitochondrial respiration mutants, atp-3 (ATP-synthase-3), which encodes a subunit of the mitochondrial ATP-synthase (194), and clk-1 (clock abnormal-1), defective in ubiquinone synthesis (51, 139), additional knockdown of autophagy genes, such as unc-51, bec-1, or atg18, suppresses the life span extension (188). This demonstration suggests that the life span regulation that corresponds with the mitochondrial respiration system is mediated by autophagy.
Mitochondria and autophagy
Mitochondrial dysfunction leads to changes in membrane potential, ROS production, ATP production, and Ca2+ homeostasis. In response to these changes, cells induce compensatory pathways, including autophagy (194). A selective elimination of damaged mitochondria by autophagy (mitophagy) might be one of the pathways utilized in response to mitochondrial dysfunction. Under nonstarvation conditions, mitophagy can be induced by mitochondrial dysfunction in yeast (89, 142). This preferential degradation of damaged mitochondria before inducing apoptotic cell death is believed to be either a mechanism for mitochondrial quality control and/or an important cytoprotective response (142). It is suggested that mitochondrial ROS production acts as a redox signal to regulate autophagy (168). Although ROS-dependent oxidative damage is associated with age-related disorders, at low concentration ROS function as signaling molecules in a number of biological processes, such as defense against invasion of microorganisms, and cell growth (20). ROS damage may induce mitochondrial permeability transition (MPT) and provide a signal leading to induction of autophagy (159). Accumulating evidence suggests that the outcomes of autophagy induction, including those mediated by ROS, can either promote cell survival or may be associated with cell death (134). In the latter case, however, it may be that the autophagic response was insufficient to prevent cell death, which thus occurs with “autophagic features.”
ROS formation following starvation, rapamycin treatment, or ischemia/reperfusion conditions is essential for autophagy induction (27, 127, 168). Several studies suggest that ROS act as a positive signal to regulate autophagy. A recent finding shows that ROS are required to induce autophagy under starvation conditions, and treatment with antioxidative agents abolish the subsequent autophagosome formation (168). Nutrient starvation leads to mitochondrial H2O2 accumulation partially through a class III PtdIns3K. A direct target for oxidation by H2O2 is Atg4, a protease essential for autophagy. Oxidation of this protein leads to inhibition of its deconjugation activity (168), which allows the accumulation of phosphatidylethanolamine-conjugated Atg8 on the autophagosome membrane, an important step in autophagosome formation (204). Based on this observation, a model for the regulation of Atg4 activity is that the H2O2 generated from mitochondria forms a gradient which results in a corresponding gradient of Atg4 activity; Atg4 closer to the mitochondria is less active compared with Atg4 further away in the cytosol, thus regulating the lipidation status of Atg8 and autophagosome formation (167). Additionally, ROS are suggested to act as a signal for mitophagy. In response to rapamycin or NGF deprivation, ROS generation and mitochondrial lipid peroxidation are suggested to induce mitophagy (91, 93).
Treatment with the caspase inhibitor zVAD promotes necrotic cell death when induced by lipopolysaccharides or cell death ligands (195, 205). Autophagy is induced in macrophages challenged with lipopolysaccharides when caspase activation is inhibited. ROS production is increased in these macrophages, and the induction of autophagy results in caspase-independent cell death (205). It is possible that, in this event, ROS might be involved in autophagy induction, because superoxide treatment results in cell death with autophagic features in these same cells. In another cell line, murine L929 fibrosarcoma, a similar event occurs when caspases are inhibited by zVAD (212). Unexpectedly, catalase is selectively degraded by autophagy upon zVAD treatment, leading to ROS accumulation in mitochondria, and ultimately cell death (213). In contrast, a recent finding suggests that autophagy plays a protective role preventing zVAD-induced cell death in L929 cells (202). In this study, autophagy induced by rapamycin or serum deprivation protects against z-VAD-induced cell death. In addition to caspase inhibition, zVAD is able to inhibit lysosomal cathepsin and calpain activities, which are required for autophagy in mammalian cells (38). The cell death reported previously might be due to the block of autophagy at a late stage through inhibition of calpain and/or other additional unknown effects of zVAD (202).
Mitochondrial dysfunction can be induced by mitochondrial ETC inhibitors. For instance, the ETC complex I and II inhibitors, rotenone and TTFA, induce cell death with autophagic features in transformed/cancer cell lines through ROS production (27). In contrast, the same ETC inhibitors fail to induce ROS generation and cell death with autophagic features in non-transformed primary mouse astrocytes (27), suggesting that induction of autophagic cell death may be a strategy for cancer treatment especially for those tumors where the cells are resistant to apoptosis. Indeed, several antitumor drugs may rely on this approach to kill cancer cells. For instance, an anti-cancer drug currently in phase II clinical trials, FK866, which targets a NAD biosynthesis enzyme to deplete intracellular NAD, is able to induce autophagic cell death in neuroblastoma (10, 11, 65, 67). Additionally, sodium selenite was demonstrated to induce cell death in several types of cancer through cell death with autophagic features, including malignant gliomas, which are resistant to apoptotic therapies. Sodium selenite can cause mitochondria membrane potential collapse, high superoxide anion generation, and induction of mitophagy (88). This cell death was blocked by overexpression of Cu-ZnSOD or MnSOD but not catalase. The fact that superoxide generator treatment alone is sufficient to induce autophagic cell death in gliomas (88) supports the idea that mitochondria could regulate autophagy through ROS production.
The antitumor effect of another chemical, arsenic trioxide (As2O3), has been shown through upregulating BNIP3 and induced autophagic cell death in malignant glioma cells (83). BNIP3 is a BH3 domain-containing pro-apoptotic Bcl-2 family protein. Augmentation of the levels of either BNIP3 or another BH3 domain-containing pro-apoptotic protein, Bax, induces autophagic cell death (192). Autophagic cell death induced by overepxression of BNIP3 or Bax is dependent on the MPT and ROS accumulation (192). All together, these data suggest that through ROS generation mitochondria regulate autophagy activity. Autophagy and mitophagy induction may contribute to both cell survival and cell death, however, it is not clear what factors lead to which fate. Understanding the mechanisms that underlie the decision for life or death mediated by autophagy would provide potential therapeutic value in treatments for cancer as well as mitochondrial-associated disease. Finally, it is important to note in some of the above situations that, although we refer to autophagic cell death, it has generally not been determined whether cell death is actually due to autophagy, and if so these situations are generally non-physiological in that they rely on various inhibitors and/or the absence of apoptosis.
Mitophagy
Mitochondria are degraded through autophagy and can be detected within double-membrane cytosolic vesicles (181). However, there have been some questions as to whether a selective form of mitochondrial degradation (mitophagy) actually occurs. The evidence for selective mitophagy comes primarily from yeast. A mitochondrial outer membrane protein, Uth1, and Aup1, a mitochondrial intermembrane space phosphatase related to mammalian PP2C, are apparently required for specific delivery of mitochondria into the yeast lysosome analog, the vacuole (22, 92, 182). Serum withdrawal promotes mitophagy of dysfunctional mitochondria or those with mtDNA mutations but spares the normal organelles in mammalian cells (56). Direct experimental evidence comes from the selective removal of depolarized mitochondria after laser-induced photodamage in living hepatocytes (100).
“Giant” mitochondria are commonly observed in aged cells, especially in long-lived post-mitotic cells, such as cardiomyocytes and skeletal muscle fibers (46). Elimination of dysfunctional mitochondria through mitophagy is proposed to retard aging (56). These senescent mitochondria are often enlarged with mtDNA mutations and protein alterations due to oxidative damage accompanied by a lack of turnover. Through fusion and fission, mitochondria can complement the damaged unit by exchange of content and restore their function, which is thought to be a defense mechanism against mitochondrial dysfunction (144). However, depolarized mitochondria fail to undergo fusion and fission events and are targeted for autophagic elimination (189). Interestingly, inhibition of autophagy results in a lower inner membrane potential, which blocks mitochondrial fusion, thus increasing the giant mitochondria accumulated in rat myoblasts and human fibroblasts (140). These findings imply that mitochondria removal by autophagy is a key mechanism for quality control.
What is the reason for the failure in mitochondria turnover by autophagy in aged cells? One explanation is the age-associated decline in mitophagy (126). The decreasing autophagy activity may provide a cellular environment allowing the accumulation of dysfunctional mitochondria. This idea is supported by the ability of anti-lipolytic agents, which mimic nutrient starvation, to stimulate autophagy, to rescue aging cells from the accumulation of dysfunctional mitochondria in rat liver (23, 43). Additionally, injection of anti-lipolytic agents in mice has an anti-aging effect by decreasing plasma insulin, free fatty acids, and glucose, and increasing autophagic activity in vivo (44). Another possibility is that some dysfunctional mitochondria fail to be recognized by the autophagy machinery and thereby accumulate with age (110).
What signals induce mitophagy for mitochondrial turnover? Some evidence suggests that the mitochondrial permeability transition caused by ROS damage initiates mitophagy as discussed above. Along this line, certain mtDNA mutations may make the organelle less prone to mitophagy degradation because the respiration defect leads to a decrease in ROS production and less oxidative damage, which might result in a decrease in the recognition signals for mitophagy (109). The MPT describes the phenomenon of the opening of nonspecific high-conductance permeability transition pores in the mitochondrial inner membrane. It is proposed that ROS cause oxidative damage to mitochondrial membrane proteins or mtDNA mutation resulting in abnormal protein synthesis, which then leads to defects in protein folding, aggregate formation, and the formation of nonspecific aqueous pores (109, 110). The opening of the pores leads to the MPT and may induce autophagy (131). At low concentration, ROS may cause mild oxidative damage and the MPT, inducing both autophagy and mitophagy and promoting cell survival (131). One example is the starvation-induced mitophagy in rat hepatocytes, which is mediated by the MPT (45). Another example suggests that mitophagy is an adaptive response that promotes cell survival by preventing ROS generation and maintaining redox homeostasis. In response to hypoxia, cells repress mitochondrial respiration by mitophagy. When MEFs are cultured under hypoxic conditions, the increasing generation of ROS by the mitochondria is necessary to activate the transcription factor, hypoxia-inducible factor 1 (HIF-1) (58). HIF-1 then induces BNIP3 expression and subsequent mitophagy induction (171). BNIP3 augmentation could increase the free Beclin 1 level for autophagy induction by competing for binding to Bcl-2 (215), which inhibits autophagy by directly interacting with Beclin 1 (150) (FIGURE 2). Similar phenomena are also observed in lungs and the lung epithelial cells of mice (215). Moreover, mitophagy also plays an essential role during development. For example, the complete elimination of mitochondria by mitophagy is required for the maturation of reticulocytes. A recent study shows that BNIP3L (NIX) is required for selective sequestration of mitochondria into autophagosomes (165, 169). Similarly, BNIP3L is also involved in mitophagy during terminal erythroid differentiation. BNIP3L knockout mice develop anemia and show mitochondrial retention, and the red blood cells have a shorter life span in vivo (165). Both BNIP3 and BNIP3L are able to induce mitochondrial depolarization, cytochrome c release, and apoptosis when overexpressed (25, 26, 73). However, BNIP3L-mediated mitophagy in reticulocytes may not depend on mitochondrial depolarization since it is independent of Bax or Bak and is not blocked by an MPT inhibitor (165, 169).
Mitophagy can be induced when apoptosis is blocked. As mentioned above, in various transformed cells that are apoptosis deficient or upon inhibition of caspase activities, mitophagy induction results in cell death (28, 42). Among those cases, ROS generation plays a role in mitophagy induction. It is proposed that the ROS level can control the balance between life and death (20). Additionally, the extent of MPT resulting from oxidative damage may lead to different cellular responses, including autophagy, apoptosis, and necrosis as shown in FIGURE 3 (89, 109). At low concentration of ROS, the stress level is just enough to lead to MPT; autophagy and mitophagy may be induced as a repair system for damaged mitochondrial removal. As the oxidative damage increases, mitochondria may no longer release factors that promote survival, and instead molecules that trigger apoptosis may leak out. Last, since the extreme oxidative stress causes severe MPT, neither autophagy nor apoptosis can progress due to ATP depletion. Thus necrosis may take place. Whether autophagy/mitophagy leads to survival or contributes to death probably depends on the severity of mitochondrial damage, the length of ROS exposure, or whether the autophagy machinery is overwhelmed by the organelle damage.

Download figureDownload PowerPoint
Conclusion
Autophagy is a major mechanism for the removal of damaged cellular structures and is critical to maintain cell survival. As demonstrated by various studies, autophagy genes are important for long-term viability. Signaling pathways, such as insulin/IGF-1, TOR, PKA, PKB, dietary restriction, mitochondrial respiration, and calorie restriction are important for life span regulation. Autophagy induction in response to these signals may promote the removal of damaged proteins or organelles and maintain proper metabolism, which is crucial for cell survival (94).
Mitochondria play an important role in aging and regulate autophagy through ROS generation and the MPT (131). Autophagy induction signaled from mitochondria can result in enhanced survival or may possibly contribute to cell death. Uncovering the molecular mechanisms underlying autophagic regulation may help us to manipulate autophagy for therapeutic purposes.